In the rapidly expanding Internet of Things (IoT) landscape, energy efficiency stands as a critical priority. IoT devices, often deployed in remote or inaccessible locations, rely on minimal power to function optimally over extended periods. Traditional power sources, such as batteries, may prove inadequate, especially when maintenance or replacement is difficult or costly. This is where energy harvesting comes into play. By tapping into ambient energy sources—like light, heat, vibration, and radio frequency (RF) signals—IoT devices can sustain themselves indefinitely. Designing analog circuits for energy harvesting requires careful consideration of multiple factors to efficiently convert these available energy sources into usable power.
Understanding Energy Harvesting for IoT
Energy harvesting is the process of capturing small amounts of energy from the surrounding environment and converting it into electrical energy. This energy is then stored or used immediately to power electronic devices. For IoT applications, energy harvesting holds immense promise, allowing devices to operate autonomously for years without the need for battery replacements or external power sources.
Ambient energy sources include solar (photovoltaic cells), thermal (thermoelectric generators), mechanical (piezoelectric materials), and electromagnetic (RF energy). Each source has unique characteristics that dictate the design approach for the analog circuits involved in harvesting and managing energy.
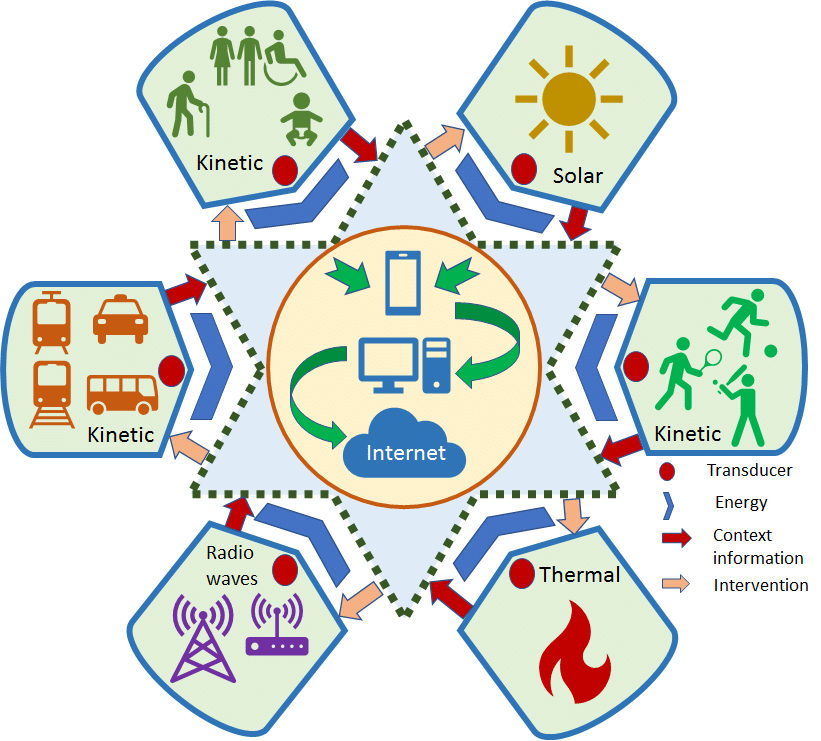
Key Requirements for Energy Harvesting Circuits
To create a successful energy harvesting circuit, you must consider several factors that influence its efficiency and reliability. It is not only about selecting the right components but also about understanding the nuances of analog design that can significantly impact performance.
Efficient Power Conversion
At the heart of every energy harvesting circuit lies the need to convert captured energy from its raw form into a usable one. This conversion process requires circuits that operate with maximum efficiency to ensure minimal power loss. When energy sources like solar, RF, or mechanical vibrations provide low and irregular energy levels, every microwatt counts.
High-Efficiency Converters: To achieve this, use highly efficient converters tailored to specific energy sources. For example, low-power boost converters, which step up the voltage from a lower input to a higher output, are ideal for low-voltage energy sources. These converters operate with minimal quiescent current, maximizing the harvested energy.
Switching Frequency and Control: The choice of switching frequency in converters directly impacts efficiency. Lower frequencies reduce switching losses, while higher frequencies allow for smaller component sizes. Striking the right balance is key, and advanced control algorithms, such as pulse-frequency modulation (PFM), help dynamically adjust the switching frequency to match the available energy levels.
Low-Voltage Operation and Startup
Many energy-harvesting sources, such as thermoelectric generators or piezoelectric transducers, produce very low voltages, often insufficient to power conventional circuits directly. To address this, analog circuits designed for energy harvesting must operate effectively at low voltage levels.
Ultralow Voltage Thresholds: Use components that function efficiently at low voltage thresholds. Choose devices like transistors and diodes that have a low forward voltage drop, which minimizes energy loss during the conversion process.
Startup Circuits: Incorporating startup circuits that can initiate operation at low voltages is crucial. Once activated, these circuits help boost the voltage to a level where the rest of the system can operate normally. Such circuits are essential in ensuring that the energy harvester begins functioning even when the initial energy availability is minimal.
Impedance Matching for Maximum Power Transfer
Maximizing power transfer from the energy source to the harvesting circuit involves ensuring impedance matching between them. Impedance matching minimizes the reflection of energy and optimizes the amount of power transferred.
Dynamic Impedance Matching Circuits: Design circuits that adapt their impedance to match that of the energy source dynamically. This approach is particularly important for sources with variable characteristics, such as solar panels that change impedance with light intensity. Employing techniques like load line optimization ensures that the maximum amount of power is extracted under varying conditions.
Noise Minimization and Filtering
Energy harvested from ambient sources often carries noise and unwanted signals that can interfere with the proper functioning of the IoT device. To maintain signal integrity, noise minimization and filtering play a vital role.
Analog Filters: Use analog filters such as low-pass, high-pass, and band-pass filters to remove noise from the harvested signals. For instance, a low-pass filter can eliminate high-frequency noise from an RF energy source, allowing the circuit to focus on the desired energy band.
Shielding and Grounding Techniques: Effective shielding and grounding techniques are also critical in preventing external electromagnetic interference (EMI) from affecting the energy harvesting circuits. Proper PCB layout practices, like minimizing loop areas and using ground planes, contribute significantly to noise reduction.
Analog Circuit Components for Energy Harvesting
Designing an energy harvesting circuit involves several key components that work together to capture, convert, and store ambient energy. Understanding the role of each component helps in creating a more efficient and reliable design.
Energy Harvesting Modules
Energy harvesting modules serve as the primary interface between the ambient energy source and the circuit. These modules include photovoltaic cells for solar energy, thermoelectric generators for thermal energy, and piezoelectric transducers for mechanical energy.
Optimizing Performance: To optimize performance, consider factors like the surface area of photovoltaic cells, the material properties of thermoelectric generators, and the resonance frequency of piezoelectric transducers. Matching these parameters with the specific energy environment enhances the overall efficiency of energy capture.
Voltage Reference Circuits
Voltage reference circuits provide a stable output voltage, which is crucial for consistent performance in energy harvesting applications. These circuits maintain a constant voltage level despite variations in the input voltage or load conditions.
Precision Voltage References: Use precision voltage references that operate with minimal current consumption. This choice ensures that the reference voltage remains stable, which is particularly important in low-power environments where fluctuations can significantly impact performance.
Energy Storage Elements
Energy storage elements, such as capacitors and rechargeable batteries, store the harvested energy and provide it to the IoT device when needed. The choice of storage depends on factors like the energy density required, the charge-discharge cycle, and the environmental conditions.
Hybrid Storage Solutions: Combining different types of storage elements can provide a balanced solution. For example, supercapacitors can deliver quick bursts of power for immediate needs, while rechargeable batteries provide long-term energy storage. This hybrid approach maximizes both short-term and long-term energy availability.
Design Considerations for Analog Circuits in Energy Harvesting
When designing analog circuits for energy harvesting, certain considerations play a pivotal role in ensuring the efficiency and reliability of the system.
Temperature Compensation
Energy harvesting circuits are often exposed to varying environmental conditions, including temperature fluctuations. These fluctuations can affect component characteristics, leading to changes in circuit performance.
Temperature Compensation Techniques: Use temperature compensation techniques to maintain consistent performance. For example, selecting resistors with low temperature coefficients or incorporating thermistors in the circuit helps counteract the effects of temperature changes.
Size and Form Factor Constraints
IoT devices are typically compact, requiring energy harvesting circuits to fit within small spaces. The size and form factor of the circuit must align with the overall device design.
Miniaturization Strategies: Adopt miniaturization strategies, such as using integrated circuits (ICs) specifically designed for energy harvesting applications. These ICs combine multiple functions, like rectification, voltage boosting, and storage management, into a single package, reducing the overall footprint of the circuit.
Environmental Adaptability
Different energy sources have unique characteristics, and the efficiency of energy harvesting can vary with environmental changes. A robust design must adapt to these variations and maintain optimal performance.
Adaptive Algorithms: Implement adaptive algorithms that modify the circuit’s behavior based on real-time environmental conditions. For example, an algorithm could adjust the maximum power point tracking (MPPT) settings of a solar energy harvester based on changing sunlight levels, ensuring continuous optimal performance.
Choosing the Right Energy Source
Before diving into the design specifics, one must carefully evaluate the ambient energy sources available for harvesting. Each source has different advantages, limitations, and efficiency factors:
- Solar Energy: Solar energy is among the most popular choices for IoT devices exposed to sunlight or indoor lighting. Photovoltaic cells convert light directly into electrical energy, providing a relatively high power output. However, the efficiency of this conversion depends on factors like light intensity, cell material, and surface area.
- Thermal Energy: IoT devices located in environments with temperature differentials can leverage thermal energy harvesting. Thermoelectric generators (TEGs) use the Seebeck effect to convert heat differences into electrical power. While thermal energy can be reliable in certain scenarios, the power output is often limited to a few milliwatts.
- Vibration and Mechanical Energy: Piezoelectric materials can convert mechanical energy, such as vibrations or pressure, into electrical energy. This approach suits IoT devices attached to machinery or vehicles, where mechanical movement is abundant. Nonetheless, the power generated from piezoelectric sources tends to be sporadic and requires efficient storage solutions.
- RF Energy: Harvesting RF energy involves capturing electromagnetic waves from the surrounding environment. RF harvesting works well in urban settings with high densities of RF signals, such as Wi-Fi, cellular, and television broadcasts. However, the energy harvested from RF sources is usually low, necessitating highly efficient power management circuits.
Designing Efficient Analog Front-End Circuits
To successfully harness ambient energy, a robust analog front-end (AFE) circuit is crucial. This circuit captures, conditions, and converts the raw energy into a form suitable for storage or immediate use by the IoT device.
Rectifiers and AC-DC Conversion
Many energy sources, such as RF and vibration, produce alternating current (AC) signals. To use this energy, the AC signals must be converted into direct current (DC). Rectifiers serve this purpose by transforming the AC input into a steady DC output.
- Full-Wave Rectifiers: For maximum efficiency, a full-wave rectifier converts both the positive and negative halves of the AC signal into DC. Schottky diodes are often chosen for rectifiers due to their low forward voltage drop, which minimizes energy loss.
- Synchronous Rectification: Synchronous rectifiers use active components like MOSFETs instead of diodes to reduce voltage drops further. While more complex, they offer higher efficiency, particularly for low-voltage energy sources.
Voltage Boosting and Regulation
After rectification, the harvested voltage may still be too low to power the IoT device or to charge its storage elements. Voltage boosting circuits step up this low voltage to a level that is usable by the device.
- Boost Converters: A boost converter is a DC-DC converter that steps up the input voltage to a higher level. These converters use inductors, capacitors, and switches to transfer energy efficiently. For ultra-low-power applications, converters with low quiescent current are essential to prevent energy loss during idle periods.
- Charge Pumps: In situations where inductors are undesirable due to size or cost, charge pumps provide an alternative method for voltage boosting. They use capacitors to transfer and boost voltage in discrete steps. While generally less efficient than boost converters, charge pumps can be more compact and suitable for space-constrained designs.
Maximum Power Point Tracking (MPPT)
To maximize the efficiency of energy harvesting, the analog circuit must operate at the Maximum Power Point (MPP) of the energy source. This point represents the optimal balance between voltage and current, where power extraction is maximized.
- MPPT Algorithms: Techniques such as Perturb and Observe (P&O) or Incremental Conductance (IC) adjust the operating point of the energy harvesting circuit to maintain operation at the MPP. Implementing MPPT requires additional analog circuitry or microcontroller support, adding complexity but significantly enhancing the power harvested from variable sources like solar and RF.
Energy Storage Elements
Energy harvested from ambient sources is often irregular and insufficient for immediate use. Hence, energy storage elements are integral to energy harvesting circuits, providing a buffer that stores accumulated energy and delivers it in a controlled manner.
- Supercapacitors: Known for their high power density and rapid charge-discharge capabilities, supercapacitors serve as excellent storage elements in energy-harvesting circuits. They provide immediate power delivery for short-term needs and withstand a large number of charge cycles, making them ideal for applications requiring frequent energy bursts.
- Rechargeable Batteries: When longer-term energy storage is necessary, rechargeable batteries (such as lithium-ion or solid-state batteries) offer a higher energy density compared to supercapacitors. However, batteries are less suited for frequent charge-discharge cycles and may require additional circuitry for battery management, including protection against overcharging and deep discharge.
Minimizing Power Losses in Analog Circuits
In any energy-harvesting application, minimizing power loss is crucial. Losses can occur due to inefficiencies in rectification, voltage conversion, and storage. To address this:
- Use Low-Loss Components: Selecting components with low forward voltage drops, minimal leakage currents, and low equivalent series resistance (ESR) can significantly reduce power loss. Schottky diodes, low-threshold MOSFETs, and high-efficiency capacitors are essential choices in low-power designs.
- Optimize PCB Layout: A well-designed PCB layout minimizes parasitic inductance and capacitance, which can degrade performance. Ensuring short and wide traces for current paths, minimizing the distance between high-current components, and proper grounding are essential practices to reduce power loss and electromagnetic interference (EMI).
- Employ Low Quiescent Current Circuits: Circuits that operate at extremely low quiescent currents help maximize the harvested energy. Quiescent current, which flows when the circuit is idle, can significantly impact the overall efficiency of energy harvesting. Opting for low-power components ensures that the circuit consumes the least possible power in standby mode.
Implementing Power Management Strategies
Energy harvesting circuits need effective power management strategies to maximize the use of the harvested energy. Several approaches ensure efficient energy use in IoT devices:
- Dynamic Power Scaling: Adjusting the operating voltage and frequency of the microcontroller or other digital components based on real-time processing requirements minimizes power consumption. This strategy ensures that the device only consumes power proportional to its workload.
- Duty Cycling: For IoT devices that do not require continuous operation, duty cycling involves periodically turning off parts of the circuit or the entire device to save energy. An effective duty cycling strategy involves maximizing sleep periods and minimizing the time the device stays active.
- Power Gating: Power gating involves shutting down parts of a circuit that are not in use, effectively eliminating leakage currents. This technique is particularly useful for analog front-end circuits that do not need to be active continuously.
Advanced Circuit Design Techniques for Energy Harvesting
To further enhance the efficiency and reliability of energy harvesting circuits, several advanced techniques can be employed:
Adaptive Control Systems
Adaptive control systems adjust circuit parameters in real-time based on environmental conditions and energy availability. For instance, a solar energy-harvesting circuit could adjust its maximum power point tracking algorithm depending on the intensity of sunlight or switch to a different energy source when one becomes unavailable.
Load Matching Techniques
For optimal energy transfer, load matching techniques ensure that the impedance of the energy source matches the impedance of the harvesting circuit. Impedance matching maximizes the power transferred from the source to the circuit, improving overall efficiency.
Energy Prediction Models
Using predictive algorithms, the energy availability from ambient sources can be forecasted based on historical data and environmental factors. Predictive models help IoT devices plan their energy consumption patterns, further optimizing their operational efficiency.
Understanding the Role of Analog Circuits in Energy Harvesting
Analog circuits play a pivotal role in energy harvesting by enabling the conversion, conditioning, and regulation of ambient energy into usable electrical power. Unlike digital circuits, which operate with discrete voltage levels, analog circuits handle continuous signals, making them ideal for interfacing with various types of energy sources.
To design effective analog circuits for energy harvesting, one must consider several key factors:
- Source Compatibility: Different ambient energy sources have unique characteristics. Analog circuits must be designed to accommodate the specific properties of the chosen energy source, such as its voltage, current, and waveform.
- Conversion Efficiency: Maximizing the efficiency of the energy conversion process is critical to ensure that the harvested energy is sufficient to power the IoT device. This involves selecting components and circuit topologies that minimize losses.
- Energy Storage and Management: Since ambient energy is often intermittent and unpredictable, it is crucial to incorporate energy storage and management strategies. These strategies ensure that the device can operate reliably, even when the energy source is temporarily unavailable.
Exploring Advanced Rectification Techniques for Energy Harvesting
Rectification is a fundamental process in energy harvesting circuits, particularly when dealing with AC sources like RF signals or piezoelectric vibrations. Traditional rectifiers, such as full-wave and half-wave diode rectifiers, convert alternating current (AC) into direct current (DC). However, to optimize performance in energy-harvesting scenarios, more advanced rectification techniques are needed.
Precision Rectifiers for Low-Voltage Applications
Many energy sources, like RF or thermal gradients, produce very low-voltage outputs. Standard diode rectifiers may not perform efficiently under these conditions due to their forward voltage drop. Precision rectifiers, often built with operational amplifiers (op-amps) and low-threshold MOSFETs, offer an effective solution.
- Operational Amplifier-Based Rectifiers: These circuits use op-amps to reduce the forward voltage drop to near zero, allowing for more efficient rectification at low voltages. This approach is particularly valuable in RF energy harvesting, where the input voltage can be in the millivolt range.
- Active Rectification Using MOSFETs: In scenarios requiring high efficiency, active rectification with MOSFETs can significantly reduce losses. Unlike diodes, MOSFETs can be controlled to switch on and off at precisely the right moments, minimizing energy loss during rectification.
Synchronous Rectification for High-Efficiency Conversion
Synchronous rectifiers use active switching elements, like MOSFETs, instead of diodes. These switches, controlled by external circuitry, operate with lower conduction losses, improving overall efficiency.
- Timing Control: Proper timing control is crucial to synchronize the switching elements with the input signal. The use of microcontrollers or dedicated timing circuits ensures that the MOSFETs turn on and off at optimal points, maximizing energy transfer.
- Adaptive Switching: Advanced designs may incorporate adaptive switching techniques that adjust the switching frequency or phase based on input conditions. This adaptability enhances performance across a wide range of operating conditions, from low-light solar cells to fluctuating mechanical vibrations.
Optimizing Power Transfer Through Impedance Matching
To achieve maximum energy transfer from the source to the load, impedance matching is essential. By matching the impedance of the energy source with the impedance of the harvesting circuit, one can maximize the power delivered to the load.
Dynamic Impedance Matching Circuits
Energy sources often exhibit varying impedance due to environmental changes. Dynamic impedance matching circuits continuously monitor and adjust the impedance to maintain optimal power transfer.
- Automatic Matching Networks: Using tunable capacitors and inductors controlled by microcontrollers, these networks dynamically adjust their impedance to match the source’s impedance. Such adaptability ensures maximum power delivery, regardless of changes in source conditions.
- Voltage-Controlled Oscillators (VCOs): In RF energy harvesting, VCOs can dynamically adjust frequency to align with the source’s impedance characteristics, enhancing overall conversion efficiency.
Advanced Power Management Strategies in Energy Harvesting Circuits
Effective power management is crucial to ensure that harvested energy is efficiently utilized by the IoT device. Several advanced strategies can be employed to optimize power consumption and extend the operational life of the device.
Load Prioritization and Sequencing
IoT devices often have multiple loads that require power, such as sensors, communication modules, and microcontrollers. By prioritizing these loads based on their importance and power requirements, one can allocate energy more efficiently.
- Load Sequencing Techniques: Using programmable switches or microcontrollers, loads can be activated or deactivated based on a predefined sequence. For example, a communication module may only be powered after sufficient energy has been harvested and stored.
- Dynamic Load Scaling: Adjusting the power supply voltage and clock frequency of various components dynamically, based on real-time requirements, reduces overall power consumption. Components operate only at the performance level necessary for the task, conserving energy for when it is most needed.
Energy-Aware Duty Cycling
Many IoT applications do not require continuous operation. Duty cycling involves periodically switching components on and off to minimize power consumption.
- Adaptive Duty Cycling Algorithms: By using sensors and predictive algorithms, IoT devices can adapt their duty cycles based on environmental conditions and energy availability. For instance, a weather station may increase its sensing interval during a storm and reduce it in stable weather, balancing performance with power consumption.
- Sleep Modes and Power Down Strategies: Incorporating various sleep modes allows different parts of the device to shut down completely or operate in a low-power state when not needed. This method, combined with efficient wake-up circuitry, can significantly extend the battery life of an IoT device.
Implementing High-Efficiency Energy Storage Solutions
Energy storage is a critical component of energy-harvesting circuits, ensuring that IoT devices remain operational even when the energy source is intermittent. Choosing the right storage elements and designing efficient storage management circuits is vital.
Hybrid Energy Storage Systems
Combining different types of storage elements, such as supercapacitors and rechargeable batteries, can offer the best of both worlds—high power density and long-term energy storage.
- Supercapacitor-Battery Combinations: Supercapacitors provide rapid charging and discharging capabilities, ideal for short bursts of energy. Batteries, with their high energy density, offer sustained power delivery. A hybrid system can utilize supercapacitors to handle peak loads while the battery maintains a steady supply.
- Intelligent Energy Balancing: Advanced circuit designs can include algorithms that dynamically balance energy between supercapacitors and batteries, depending on the load requirements and the availability of harvested energy. Such designs prevent overcharging and deep discharging, prolonging the lifespan of storage elements.
Energy Routing and Distribution Networks
To manage multiple energy sources and storage elements, energy routing and distribution networks are essential. These networks ensure that harvested energy is efficiently directed to the most critical components.
- Multi-Source Harvesting Controllers: These controllers can simultaneously interface with multiple energy sources, such as solar, thermal, and RF, selecting the most efficient source at any given moment. Advanced controllers even blend power from different sources to maximize overall energy availability.
- Smart Distribution Logic: By integrating analog multiplexers and digital controllers, smart distribution circuits can prioritize which storage elements receive energy first and which loads are powered, ensuring optimal use of available resources.
Leveraging Innovative Circuit Topologies for Enhanced Performance
To push the boundaries of energy harvesting, innovative circuit topologies can offer improved efficiency, compactness, and versatility.
Switched-Capacitor Converters
Switched-capacitor converters offer an alternative to traditional inductive converters by using capacitors to store and transfer energy. These converters can be highly efficient, particularly in low-power applications where inductors may introduce excessive losses.
- Charge Redistribution Techniques: Switched-capacitor converters utilize charge redistribution methods to adjust output voltage levels without significant energy loss. This approach allows for fine-grained control of voltage regulation, making it ideal for IoT devices that operate across varying voltage ranges.
- Dual-Mode Converters: These converters can switch between capacitive and inductive modes, depending on the energy source characteristics and load requirements. By dynamically choosing the most efficient conversion method, dual-mode converters enhance overall performance.
Multi-Stage Energy Harvesting Architectures
Multi-stage architectures divide the energy harvesting process into distinct stages, each optimized for a specific task—rectification, boosting, regulation, and storage.
- Cascaded Conversion Stages: By cascading multiple conversion stages, energy loss at each stage can be minimized. For example, an initial stage may focus on maximizing the conversion of low-voltage AC to DC, while subsequent stages optimize for voltage boosting and regulation.
- Parallel Processing Stages: In scenarios where multiple energy sources are available, parallel processing stages can independently handle different sources, merging their outputs to provide a stable power supply. This approach ensures resilience and redundancy, crucial for mission-critical IoT applications.
Future Trends in Energy Harvesting for IoT
The field of energy harvesting continues to evolve, driven by advances in materials science, nanotechnology, and circuit design. Emerging technologies like flexible solar cells, triboelectric nanogenerators, and hybrid energy harvesters promise to provide more efficient, versatile, and compact solutions for IoT applications. As these technologies mature, the potential for self-sustaining, maintenance-free IoT devices grows exponentially.
Conclusion: Harnessing Energy for Sustainable IoT Devices
Designing analog circuits for energy harvesting in IoT devices requires a deep understanding of both the characteristics of available energy sources and the requirements of the target application. By leveraging efficient rectification, voltage boosting, storage management, and power minimization techniques, engineers can develop energy-harvesting solutions that prolong the life of IoT devices and reduce their environmental impact. As the IoT ecosystem expands, energy harvesting will play an increasingly critical role in enabling sustainable, long-term deployments. Thus, mastering the art of designing efficient analog circuits for energy harvesting becomes a key competency for any IoT engineer aiming to build the next generation of smart, autonomous devices.
To have a better understanding of IoT Protocols, we recommend using Smowcode to boost your productivity and create great products 10x faster.
Try Smowcode for free and Boost your Productivity by 10x. : https://smowcode.com/
Do go through our other blogs to understand IoT concepts: https://blog.smowcode.com/smart-connectivity-wi-fi-in-the-iot-era/
Link to Modbus Blog: https://blog.smowcode.com/understanding-modbus-in-industrial-iot/